The 2017 U.S. FDA approval of the first gene therapy for a genetic disease launched a promising new cycle of innovation in ophthalmic therapies.1 The retina is a prime target for gene therapy research given the large number of monogenic disorders, accessibility to target cell delivery, the noninvasive ability to monitor for disease progression or therapeutic response as well as relative immune-privilege that limits inflammatory response.2-4 However, beyond inherited retinal dystrophies (IRDs), gene therapy is even being explored to treat common chronic disorders, such as neovascular AMD, geographic atrophy, uveitis and some corneal dystrophies.
This article reviews some of the basic principles of gene therapy, as the ophthalmology community prepares for the future.
GENETIC TESTING
With more than 260 genes known to cause IRDs,5 a basic understanding of genetic testing is essential to remain current. Genetic testing is recommended by the AAO when clinical findings indicate retinal dystrophy that is possibly associated with genetic mutations.6 Genetic testing can confirm suspicion of an IRD, provide an accurate diagnosis and information about prognosis and management and assist in counseling of families, including assessing risk in family members.7
Unfortunately, ordering and interpretation of genetic test results is complex, but a facility with the basics of interpretation is useful. While single-gene testing is inexpensive, it requires a working clinical diagnosis and, consequently, has limited utility in many cases, given the nonspecific nature of clinical findings in many IRDs.8 Gene panels are more commonly used, and generally focused on diagnoses associated with multiple genes.8 Whole genome/exome sequencing is the most comprehensive but generates a high number of variants of uncertain significance (VUSs).8
Genetic testing results are not binary and involve a ranking system of each identified mutation based on standards released by American College of Medical Genetics and Genomics (pathogenic, likely pathogenic, VUS, likely benign, benign).9 Pathogenicity is determined by multiple factors, including the effect on gene coding, protein structure/function, variant association with disease in the population and in vitro/in vivo functional studies.9 VUSs can be especially difficult to address. A VUS involves a mutation variant for which there is a lack of affirmative data of pathogenicity or non-pathogenicity. A VUS may be reclassified in the future as more information accumulates.
Genetic counselors can assist patients in selecting genetic tests, interpretation of genetic test results and family planning. Fortunately, insurance can cover their services, and some providers are available for patients via a telemedicine interaction.
TYPES OF GENE THERAPY
The major categories of gene therapy include:
- Gene augmentation — adding a gene to a cell
- Gene editing — revising the existing genetic code
- Gene inactivation — silencing a gene, often a dominant negative one
- Selective toxicity — as in chimeric antigen receptor (CAR)-T cells to recognize cancer cells
- RNA therapeutics — targeting RNA, instead of DNA within the gene
Ocular gene therapy has mostly involved gene augmentation. Recessive single-gene disorders are the most amenable to gene augmentation, because the mutations causing the disease generally lead to loss-of-function of the gene product and, therefore, total absence or near absence of functional protein. In these cases, gene augmentation can correct the lost function by delivery of the normal gene. Also, in some instances, restoring a small percentage of the normal level of the gene product is sufficient to revert the phenotype.
The majority of gene therapies to treat inherited ocular diseases target autosomal recessive diseases. For example, RPE65 mutation-associated retinal dystrophy is a recessive monogenic disorder in which Luxturna (Spark Therapeutics), the only FDA-approved ocular gene therapy, corrects the lost function. In contrast, gain-of-function mutations produce dominant phenotypes. These diseases may be less amenable to gene therapy treatment, as one gene copy expresses an abnormal product that has to be suppressed while the other expresses a protein that functions normally.10 Consequently, developing a gene therapy for autosomal dominant RP has been more challenging.
In addition to addressing genetic mutations in native genes, gene therapy can be utilized in a “biofactory approach” to produce non-native proteins. In neovascular AMD, gene therapy is already being assessed using a biofactory approach to chronically express anti-angiogenic proteins such as pigment epithelium-derived factor, soluble fms-like tyrosine kinase-1 (sFLT-1) as well as aflibercept (Eylea, Regeneron), ranibizumab (Lucentis, Genentech), angiostatin and endostatin. The complement system is also a target for gene therapy in AMD. In humans, deposition of membrane attack complex in Bruch’s membrane and choriocapillaris increases significantly with aging and with AMD,11 and some gene therapies are being developed to inhibit components of the complement cascade.
Another gene therapy approach involves optogenetic technology that may render retinal ganglion cells responsive to light. This therapy comprises a vector encoding a photosensitive protein, belonging to the subfamily of channelrhodopsins, which functions as sensory photoreceptors in green algae. Once this protein is expressed, it confers a photoreceptor-like function to the target ganglion cells. As noted above, an additional form of gene therapy is RNA therapeutics, which involves targeting RNA, instead of DNA within the gene. Current trials are assessing intravitreal antisense oligonucleotides in IRD.
DELIVERY METHODS
A variety of viral and non-viral gene delivery methods have been developed over the past two decades.
The viral vectors most extensively used include the adenovirus, adeno-associated virus (AAV), gamma-retrovirus and lentivirus. The choice of viral vector is specific to each application and depends on a combination of factors, such as tissue tropism, cloning capacity of the vector (which determines the size of the expression cassette that can be accommodated in the genome of the virus) and safety concerns (inflammatory responses and genotoxicity/insertional oncogenesis).
AAVs are small, single-stranded DNA viruses of the parvovirus family (See Figure).12 AAV vectors transduce quiescent tissues, and their genome is primarily maintained as extrachromosomal monomeric and concatemeric circles. More than 100 different AAV serotypes have been described, each of them displaying enhanced tropism for a specific set of tissues. To date, AAV is the most common viral vector utilized in retinal genetic therapy. AAV serotypes 2, 5 and 8 have been commonly used in retinal gene therapy applications, transducing photoreceptors and/or retinal pigment epithelium (RPE). Multiple features make it an excellent vector choice for these diseases, including the non-integrating nature, low inflammatory potential, low immunogenicity, low retinal toxicity at appropriate doses, non-pathogenic nature, ability to transduce non-dividing cells and excellent track record of safety in human trials.13 AAV vectors do have limitations, which include having a restricted transgene capacity (4.5-5.0 kb) and the risk of being eliminated by the humoral immune response in patients who have previously been exposed to the virus.14 However, the risk for immunogenicity with AAV vectors is low when targeting relatively immune-privileged tissues such as the retina.15
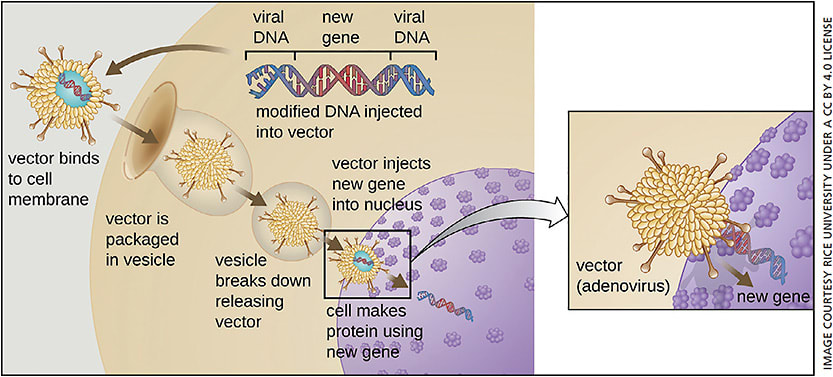
Lentiviruses are RNA viruses of the retrovirus family. Commonly used lentiviral vectors derive from the human immunodeficiency virus 1 (HIV1) or the equine infectious anemia virus (EIAV).16 Lentivirus vectors efficiently integrate their genome into the host cell genome and do not require cell division for integration.17 An added attractive feature is the cloning capacity, up to 10 kb.18 Lentiviruses have recently become a popular choice because they have improved safety features relative to gamma-retroviruses, as the vector genome does not preferentially integrate in the proximity of oncogenes.19
Other nonviral delivery methods are being investigated and have potential for titratable and repeat dosing according to clinical need. In addition to antisense oligonucleotides, DNA-compacted nanoparticles, which are single DNA molecules, compacted polyethylene glycol-substituted polylysine 30-mers (CK30PEG) have been explored. This nonviral gene therapy technique would incur less risk of immune response in patients with preexisting viral immunity and can transfer large genes beyond the carrying capacity of AAV or even lentivirus.
The safety and potential utility of the nanoparticles has been demonstrated in the lung, with introduction of the cystic fibrosis transmembrane conductance regulator through an intranasal route in a Phase 1 clinical trial.20 In the retina, DNA nanoparticle-mediated ABCA4 delivery has been shown to rescue several IRDs, including Stargardt dystrophy in mouse models,21 and reporter genes have been expressed in RPE as long as two years.22
Since DNA nanoparticles have been found to have lower transduction efficiency per vector genome than AAV, higher vector amounts may be compensated and repeated dosing may be required after one to two years, which would not be practical via vitrectomy with subretinal injection.23 However, intravitreal or even suprachoroidal administration could circumvent this problem, as these routes are conducive to repeat minimally invasive treatment. As with intravitreally-administered antisense oligonucleotides, this paradigm could facilitate titration to clinical response or need, as opposed to a one-time treatment with chronic protein expression using current viral vector-based techniques.
PRODUCTION OF GENE THERAPY
Viral gene therapies are complex, requiring more complicated processing than pharmaceuticals because virus capsids are developed within cell lines. Unlike nonviral gene therapies such as DNA nanoparticles or oligonucleotides, cell lines serve as “biofactories” to produce viral vectors.
With AAV2, for example, these cell lines typically undergo “triple transfection” with three plasmid constructs carrying expression cassettes encoding the therapeutic transgene (including regulatory elements such as promoters and enhancers), the AAV (rep and cap genes) and helper virus sequences (to replicate and pack the recombinant AAV with therapeutic transgene). Multiple purification steps ensue to extract the viral capsids from the cell media.
ADMINISTRATION OF OCULAR GENE THERAPY
Vector delivery to the target retinal tissue involves several potential methods. The most commonly investigated method involves pars plana vitrectomy followed by retinotomy and injection of the viral vector with genetic material into the subretinal space. This method creates a temporary retinal detachment but allows for direct delivery to the cells of interest. The virus then “infects” or enters the retinal pigment epithelial cells or photoreceptors, ultimately causing the host cell’s own translational machinery to express the protein.
Alternatively, injection of the vector into the vitreous cavity has been attempted. Although this method may be less invasive and potentially have fewer procedure-related complications, the penetration of viral vector to the target tissue has historically been perceived as inferior to that of subretinal injections.24 Additionally, animal models have demonstrated an induced humoral immune response to intravitreally delivered viral vectors that was not observed with subretinally delivered viral vectors. However, techniques such “directed evolution” of viral vectors may enhance intravitreal delivery, and at least one such promising intravitreally administered vector employing an anti-VEGF biofactory approach is currently in clinical trial. Furthermore, clinic-based intravitreal or suprachoroidal delivery may circumvent some of the logistic issues of operating room-based subretinal delivery.
OPTIMIZATION AND REGULATION
Beyond selection of vector, numerous factors are involved in optimizing gene therapy. Within the vector, the gene is actually complementary DNA or cDNA with no introns, only the coding exons. Codon optimization involves the introduction of synonymous mutations into recombinant genes, changing rare codons to common codons to improve protein translation efficiency. Also, there are regulatory elements such as promoters and enhancers, which partially determine where and how robustly the gene is expressed as well as optimization of the translational start site (eg, Kozak sequence).
In addition to optimizing the vector, the gene itself, regulatory elements, delivery method and location, an immunomodulatory regimen may be used to limit the risk of immune response. These parameters all factor into differentiation, in which two gene therapies carrying the same transgene may have differing safety and efficacy profiles.
In the future, there may be “regulate-able” gene therapy, in which small, orally administered molecules turn protein production on and off as needed. For example, preclinical studies have demonstrated the efficacy of a tetracycline-responsive “OFF-type” riboswitch in regulating aflibercept expression in the mouse retina following AAV delivery of a gene encoding aflibercept.25 Transgene regulation by physiological condition may be possible in the future. One group developed a hypoxia-regulated gene therapy (using hypoxia inducible factor-1 response elements) to drive expression of endostatin in the same hypoxic/inflammatory conditions that drive VEGF expression and growth of choroidal neovascularization in a murine model.26
CONCLUSION
Gene therapy has proven to be relatively safe in a multitude of small clinical trials. With the potential for continued optimization and rapid therapeutic innovation in gene therapy, ophthalmology has an exciting future. However, to remain current, ophthalmologists must understand genetic testing and the basic aspects of gene therapy. OM
REFERENCES
- LUXTURNA (voretigene neparvovec-rzyl) December 19, 2017 Approval Letter. 2017, US Food & Drug Administration: Silver Spring, MD.
- Streilein JW. Ocular immune privilege: therapeutic opportunities from an experiment of nature. Nat Rev Immunol. 2003;3:879-889.
- Takahashi VKL, Takiuti JT, Jauregui R, Tsang SH. Gene therapy in inherited retinal degenerative diseases, a review. Ophthalmic Genet. 2018;39:560-568.
- Boye SE, Boye SL, Lewin AS, et al. A comprehensive review of retinal gene therapy. Mol Ther. 2013;21:509-519.
- RetNet: Summaries of Genes and Loci Causing Retinal Diseases. September 18, 2018 [cited 2018 January 16]; https://sph.uth.edu/retnet/sum-dis.htm .
- Duncan JL, Bernstein PS, Birch DG, et al. Recommendations on Clinical Assessment of Patients with Inherited Retinal Degenerations - 2016. 2016 [cited 2017 October 3]. https://www.aao.org/clinical-statement/recommendations-on-clinical-assessment-of-patients .
- Role of Genetic Tests in IRDs and RED. [cited 2018 October 3]; http://www.retina-international.org/role-genetic-tests-irds-and-red .
- Lee K, Garg S. Navigating the current landscape of clinical genetic testing for inherited retinal dystrophies. Genet Med. 2015;17:245-252.
- Richards S, Aziz N, Bale S, et al. Standards and guidelines for the interpretation of sequence variants: a joint consensus recommendation of the American College of Medical Genetics and Genomics and the Association for Molecular Pathology. Genet Med. 2015;17:405-424.
- Sengillo JD, Justus S, Tsai YT, et al. Gene and cell-based therapies for inherited retinal disorders: An update. Am J Med Genet C Semin Med Genet. 2016;172:349-366.
- Mullins RF, Schoo DP, Sohn EH, et al. The membrane attack complex in aging human choriocapillaris: relationship to macular degeneration and choroidal thinning. Am J Pathol. 2014;184:3142-3153.
- Carter PJ, Samulski RJ. Adeno-associated viral vectors as gene delivery vehicles. Int J Mol Med. 2000;6:17-27.
- Daya S, Berns KI. Gene therapy using adeno-associated virus vectors. Clin Microbiol Rev. 2008;21:583-593.
- Surace EM, Auricchio A. Versatility of AAV vectors for retinal gene transfer. Vision Res. 2008;48:353-359.
- Hareendran S, Balakrishnan B, Sen D, et al. Adeno-associated virus (AAV) vectors in gene therapy: immune challenges and strategies to circumvent them. Rev Med Virol, 2013;23:399-413.
- Everson EM, Trobridge GD. Retroviral vector interactions with hematopoietic cells. Curr Opin Virol, 2016;21:41-46.
- Sakuma T, Barry MA, Ikeda Y. Lentiviral vectors: basic to translational. Biochem J. 2012;443:603-618.
- Kumar M, Keller B, Makalou N, Sutton RE. Systematic determination of the packaging limit of lentiviral vectors. Hum Gene Ther. 2001;12:1893-1905.
- Zufferey R, Dull T, Mandel RJ, et al. Self-inactivating lentivirus vector for safe and efficient in vivo gene delivery. J Virol. 1998;72:9873-9880.
- Konstan MW, Davis PB, Wagener JS, et al. Compacted DNA nanoparticles administered to the nasal mucosa of cystic fibrosis subjects are safe and demonstrate partial to complete cystic fibrosis transmembrane regulator reconstitution. Hum Gene Ther. 2004;15:1255-1269.
- Han Z, Conley SM, Makkia RS, et al. DNA nanoparticle-mediated ABCA4 delivery rescues Stargardt dystrophy in mice. J Clin Invest. 2012;122:3221-3226.
- Koirala A, Makkia RS, Conley SM, et al. S/MAR-containing DNA nanoparticles promote persistent RPE gene expression and improvement in RPE65-associated LCA. Hum Mol Genet. 2013;22:1632-1642.
- Han Z, Conley SM, Makkia R, et al. Comparative analysis of DNA nanoparticles and AAVs for ocular gene delivery. PLoS One. 2017;:e52189.
- Yu-Wai-Man P. Genetic manipulation for inherited neurodegenerative diseases: myth or reality? Br J Ophthalmol. 2016;100:1322-1331.
- Reid CA, Nettesheim ER, Connor TB, et al. Development of an inducible anti-VEGF rAAV gene therapy strategy for the treatment of wet AMD. Sci Rep. 2018.8:11763.
- Biswal MR, Prentice HM, Smith GW, et al. Cell-specific gene therapy driven by an optimized hypoxia-regulated vector reduces choroidal neovascularization. J Mol Med (Berl). 2018;96:1107-1118.