Cataract Surgery: Improving Overall Quality of Life
Extraction can improve health as well as vision.
By Patricia L. Turner, MD and Martin A. Mainster, PhD, MD, FRCOphth
Cataract surgery improves vision and therefore quality of life. As critical as good vision is to maintaining independence, cataract extraction serves an equally valuable purpose: it increases the light available to retinal ganglion photoreceptors (RGPs). Bright, properly timed light exposure profoundly influences human health and psychology.1-5 Bright morning light enhances the early cortisol surge, facilitating the transition from sleep to daytime physiology. Bright daytime light improves mood, reduces depression and increases that evening's sleep quality. These processes are all guided by information on environmental light levels provided by RGPs.2-4
Cataract and age-related crystalline lens yellowing reduce retinal illuminance, especially in the short wavelength (violet and blue) end of the spectrum needed by RGPs.1-5 Thus, it's no surprise that cataract surgery has many potential benefits beyond improving vision.1-5 Here's why.2, 3
Retinal Ganglion Photoreceptors
The discovery of inner retinal RGPs in 2002 revolutionized scientific understanding of retinal photoreception.6,7 Previously, outer retinal rods and cones were incorrectly believed to be the source of all human photoreception. The primary function of RGPs is gross detection of environmental light.2,3 Their input is used by nonvisual brain centers to optimize physiology.2,3
Approximately 3,000 RGPs with large receptive fields form a light-sensitive network that spans each retina.8 They require bright illuminances to function properly.2,3 Their brightness thresholds are much higher than those of cones, approximating the level of light at dawn. Retinal ganglion photoreceptors express the blue-light sensitive photopigment melanopsin in their somas, dendrites and the intra-retinal portions of their axons.9 RGPs use an invertebrate form of photopigment regeneration that does not require retinoid transport to and from the retinal pigment epithelium.10
Rods and cones subserve primarily conscious vision whereas newly discovered RGPs mediate a diverse array of immediate or delayed nonvisual responses (Figure 1). Retinal ganglion photoreception is unconscious, so we cannot recognize deficits.2,3 Rods, cones and RGPs act together in some photic responses, such as the pupillary light reflex. Rod and cone photoreceptors provide fast pupillary responses, whereas RGPs are responsible for sustained post-illumination responses to bright, prolonged light exposures.11,12
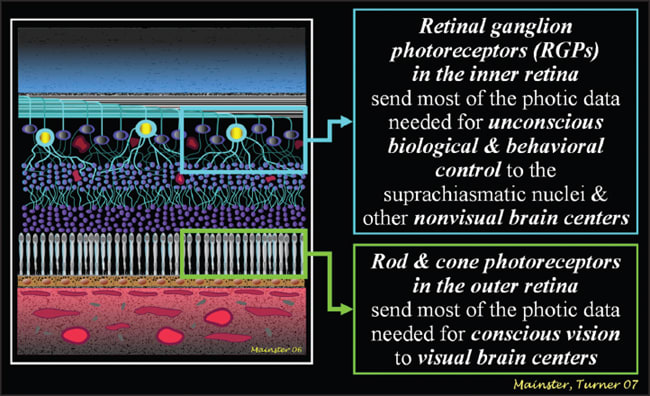
Figure 1. The brain receives photic information from the inner as well as the outer retina.6,7 Rods and cones initiate conscious vision. RGPs provide the data needed for unconscious biological and psychological controls and for bright sustained visual light levels.1-3,12 All images courtesy of Patricia L. Turner, MD and Martin A. Mainster, PhD, MD, FRCOphth
Retinal ganglion photoreceptor axons form a unique neural pathway called the retinohypothalamic tract. It transmits information on ambient illumination to more than a dozen brain centers, including the olivary pretectal nucleus involved in the pupillary light response and the paired hypothalamic suprachiasmatic nuclei (SCN), which are the human body's master biological clock.9,12 The SCN orchestrate circadian rhythms, which optimize physiology, neurobiologic behavior and hormonal secretion for anticipated activities over the daily cycle (Figure 2).2,3 Internal biologic time must be matched to external environmental time for proper physiological function. This synchronization (photoentrainment) depends critically on RGP photoreception.2,3
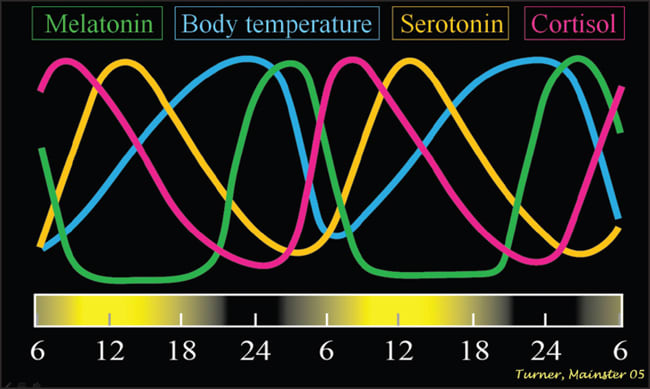
Figure 2. Virtually every non-retinal physiological, biochemical and hormonal process in the human body depends on active SCN circadian control.1-3
Disadvantages of Circadian Disruption
The suprachiasmatic nuclei utilize the autonomic nervous system, hormonal secretion and direct neural pathways to execute their physiological directives (Figure 2).3 Environmental light exposure cues can assure that the SCN's periodicity is well aligned with the 24-hour environmental day. If RGP photoreception is inadequate, however, SCN escape their proper entrainment and revert to their own intrinsic period, which usually differs from 24 hours. The SCN's endogenous circadian period is inherited, precise and unvarying with age. It ranges from 22.5 to 25.5 hours and averages 24.2 hours, so the SCN typically require an average of 12 minutes of clock resetting each day.2,3
Repeated cycling of the SCN without regard for environmental time is termed “free-running.”2,3 SCN free-running is equivalent to a watch running too fast or slow. Timing errors compound daily. Free-running biologic rhythms march in and out of alignment with geophysical time over days to months. When biologic night occurs during the geophysical day, the SCN activate sleep centers and ill-timed daytime melatonin secretion reduces alertness. People are sleepy during work hours, find napping irresistible, and suffer intractable nighttime insomnia because circadian alerting keeps them awake during the geophysical night.2,3 Biological and geophysical cycles eventually realign before again separating.
SCN dysfunction is termed chronodisruption.2,3 Chronodisruption has many forms, including free-running due to light deficiency or blindness. Classic symptoms of chronodisruption include insomnia, daytime sleepiness, fatigue, depression and cognitive deficits. They occur in shift work, jet lag, aging, light deficiency and blindness.
Most visually blind individuals have abnormal circadian rhythms because of absent or insufficient RGP photoreception.2,3 Many blind individuals describe their chronic extreme chronodisruption as being almost as disabling as their visual loss. All people with bilateral enucleation free-run because they have no photoreceptors to align their SCN to geophysical time.
Some types of visual blindness involve only rod and cone photoreceptors or visual brain centers, however, so RGP photoreceptors and nonvisual brain centers may function normally. Cortical blindness exemplifies visual blindness with normal RGP photoreception. Cortically blind patients can have normal circadian entrainment if they receive sufficient environmental light. Thus, the term “blindness” should be divided into (1) “visual-only blindness” for people who retain their RGP photoreception despite their complete loss of rod or cone photoreception and (2) “total blindness,” for people without any photoreception.2,3
Numerous studies document that visual loss and blindness are strongly statistically associated with reduced longevity.2,3 Life expectancy varies directly with visual acuity and age at onset of vision loss. Even mild visual impairment increases the risk of death more than twofold.13 Growing evidence implicates chronic chronodisruption from deficient retinal ganglion photoreception as the cause of this association.2,3
The SCN play a critical role in daily physiology.2,3 Their dysfunction produces diverse morbidities. All nucleated cells have circadian clocks similar to those in the SCN to control the timing of their daily function. Peripheral cellular clocks quickly become desynchronized, however, without the temporal alignment provided by the SCN14 (Figures 3a and 3b). Temporally disorganized cells in tissues and organs produce metabolic disarray and biochemical imbalance, activating the stress cascade.3
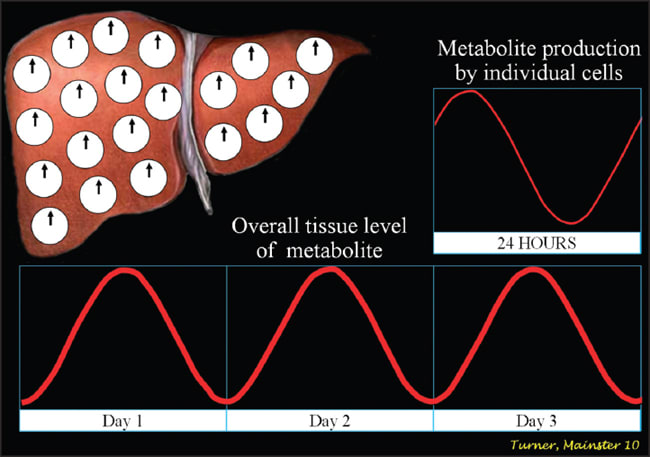
Figure 3a. With robust suprachiasmatic nuclear output, peripheral cellular clocks in tissues and organs are well aligned and serum levels of metabolites have prominent diurnal rhythms.3,14
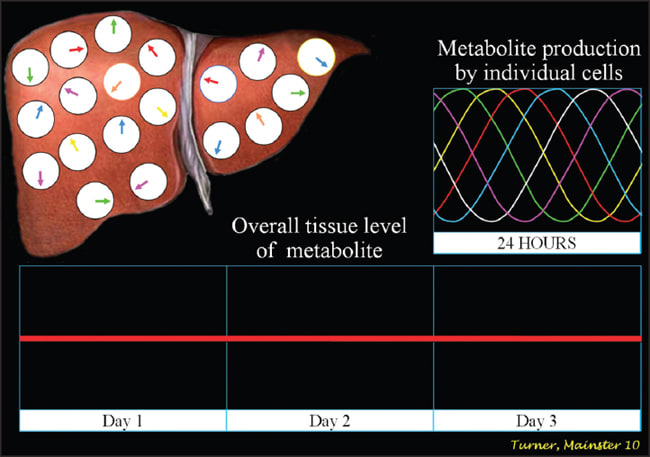
Figure 3b. Without effective suprachiasmatic nuclear signaling, peripheral cellular clocks in tissues and organs become misaligned and serum levels of metabolites lose their diurnal rhythmicity.3,14
SCN function is enhanced by exposure to bright, properly timed light.2,3 Deficient light input dampens their rhythmicity, weakening their ability to restrain the stress cascade, which results in a viscous cycle of progressively higher baseline cortisol levels.3 Chronic glucocorticoid elevation is highly neurotoxic. It is especially harmful to the hippocampus, a key memory center. Chronically elevated cortisol can decrease hippocampal volume, cause cognitive decline and increase the risk of Alzheimer's disease. Hypercortisolemia, however mild, contributes to insulin resistance. It also causes hyperactivation of the sympathetic nervous system, releasing catecholamines, which:
(1) increase cardiovascular risks,
(2) produce hyperarousal resulting in insomnia,
(3) cause depression from reduced brain serotonin levels, and
(4) increase inflammation due to pro-inflammatory cytokine release.3
Totally blind individuals can be entrained by daily exogenous melatonin taken nightly at the same time before bedtime.2,3 This therapy eliminates free-running, improves quality of life, and may increase longevity by resolving chronodisruption and its endocrinological abnormalities, including elevated catecholamines and glucocorticoids.3
Advantages of Bright Light
Bright light, which stimulates RGP input to nonvisual brain centers, has numerous systemic benefits.2,3 Shorter wavelengths are most effective because human RGP photoreception peaks at 460 nm in the middle of the blue part of the spectrum.15-17 Bright light is the most important cue for aligning SCN rhythms with external time. Brighter, longer, bluer exposures produce the most effective SCN clock entrainment because of the spectral sensitivity of RGPs and neural processing of their output.2,3
Effective light exposure enhances SCN function.2,3 SCN control the sleep-wake cycle and their robust signaling reduces daytime sleepiness while enhancing sleep quality. Bright daytime light increases nocturnal serum melatonin levels for adults of all ages.
Sleep disorders are a common aging problem, becoming chronic in more than 50% of adults over 50 years of age.2,3 Light therapy is an effective treatment for insomnia, increasing sleep efficiency, total sleep time and the duration of restorative slow wave sleep. Bright daytime light exposure allows older insomniacs to regain youthful nocturnal melatonin amplitudes and resolves their sleep complaints.18 Melatonin is only one of the many hormones that the SCN control.3 Bright light exposure improves SCN function, which in turn restores normal amplitudes of other hormones and biorhythms, including body temperature.2,3
Natural light enhances cognition, work performance and accelerates learning.2,3 Bright light increases feelings of well-being and optimism in people of all ages without depression. It immediately increases brain serotonin, depending on its spectrum and intensity. Bright light reduces the incidence of depression. Light therapy is effective for treating both seasonal and non-seasonal depression with response rates exceeding 75%.3
Light Deficiency and Ocular Aging
Inadequate environmental illumination dampens SCN function and causes chronodisruption.2,3 Three and a half billion years of evolution have produced retinal ganglion photoreceptors tuned to light with the intensity and spectrum of daylight. Typical residential or commercial artificial light is far too dim and blue-light-deficient for optimal or even effective circadian function.2,3 Humans in industrialized societies still need exposure to natural light. They are disadvantaged by its absence.2,3
Astronauts free-run at typical space shuttle illuminances below 80 lux, causing insomnia and impaired neurobehavioral performance. Young adults free-run when restricted to artificial lighting less than 200 lux. Residential illumination averages 200 lux or less, compared to 10,000 lux outdoors on dull, overcast days or during light therapy for seasonal depression. Mid-winter insomnia and seasonal affective disorders occur during winter months at higher latitudes in up to 80% and 40% of populations, respectively. They would not occur if standard room lighting in industrialized societies were adequate for RGP input to the SCN. It is not.2,3
SCN performance cannot be measured directly in humans, but SCN suppress production of the nocturnal hormone melatonin immediately when RGPs are exposed during the biologic night to light of sufficient intensity, spectrum and duration.2,3 Melatonin suppression testing is the most widely accepted method for determining the brightness, spectrum and duration of light required for SCN response to retinal ganglion photoreception. Indeed, light exposures must be sufficient to produce melatonin suppression in order to induce other nonvisual human photoreceptive phenomena such as alerting, enhanced cognition, increased heart rate, improved mood, entrainment phase shifting and superior sleep quality.3
Age-related crystalline lens yellowing and pupillary area (miosis) progressively reduce retinal illuminance from environmental light, especially in the blue part of the spectrum needed for effective RGP input to the SCN (Figure 4).1-3 The retinal illuminance needed for circadian photoreception is maximal in 10-year-olds. It declines to only 50%, 37% and 17% of that peak by ages 45, 55 and 75 years, respectively. To compensate for age-related retinal illuminance losses, light sources must be two-, three- or sixfold brighter for 45, 55 or 75 year olds, respectively. In essence, older adults need more light to maintain youthful circadian light exposures or encounter resultant losses in SCN function.2,3
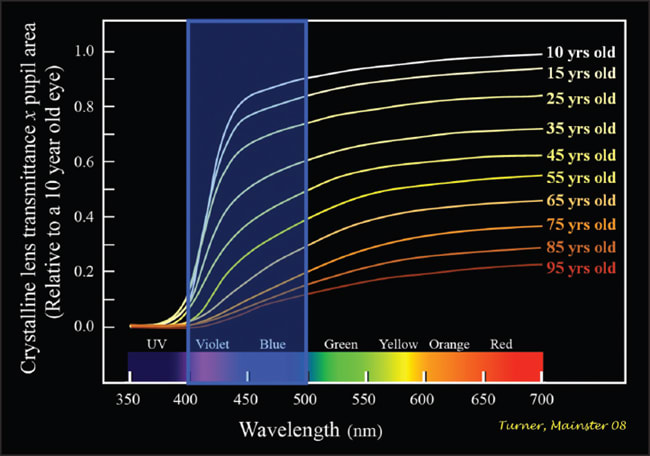
Figure 4. Retinal illumination declines progressively with age, particularly in the short wavelength violet and blue part of the spectrum essential for retinal ganglion photoreception.2,3
The significance of these age-related losses was demonstrated in a study comparing nocturnal melatonin suppression in two groups of women with average ages of 24 and 57 using optimal 460 nm monochromatic blue light and dilated pupils.19 The older women with more crystalline lens yellowing had no mela-tonin suppression in response to blue light exposures that significantly suppressed melatonin in the younger group.
Both groups equally suppressed melatonin in response to green wavelengths, demonstrating that it was the selective blocking of blue light by three decades of additional crystalline lens yellowing that made the older group insensitive to optimal RGP light exposure. Ocular aging is probably a significant contributor to progressive age-related incidences of insomnia, cognitive dysfunction, insulin resistance, hypercortisolemia, depression and flattened hormonal production.2,3
Cataract Surgery and Health
Circadian disruption is associated with morbidities often assumed to be age-related inevitabilities.2,3 Circadian amplitudes diminish when retinal ganglion photoreceptor input to nonvisual brain centers decreases due to environmental light deficiency or ocular aging. Cataract surgery increases ocular light transmittance, thereby improving retinal ganglion photoreception.1-5 Cataract surgery with clear UV-blocking IOLs has been shown to decrease insomnia and increase daytime alertness.20,21 All cataract surgery improves circadian photoreception, but colorless UV-blocking IOLs provide circadian photoreception 15-20 years more youthful than yellow-tinted blue-blocking IOLs.2,3,22,23
Femtosecond laser photolysis is being studied experimentally to increase retinal illuminance by bleaching the yellowish discoloration of aging crystalline lenses.24 This method potentially delays the need for cataract surgery by providing three to seven years of crystalline lens rejuvenation,24 consistent with our own estimates.2 Its potential use for bleaching yellow chromophores in blue-blocking IOL materials was not investigated.
Ocular aging is not a lens transmittance zero-sum game.23 Optimal cataract surgery should compensate not merely for the crystalline lens' loss of optical transparency, but also for:
(1) age-related loss in pupillary area which progressively reduces retinal illuminance
(2) progressive age-related loss of rod and retinal ganglion photoreceptors, and
(3) the decreased environmental illumination of most people in industrialized society, especially older adults.
Compelling and growing evidence now suggests that ophthalmologists improve health as well as vision with cataract surgery.1-5 Poor performance on disability glare testing has been used for decades as a relative indication for cataract surgery in patients with visual complaints despite good high contrast visual acuity.25 Effective documentation of circadian photoreception losses may also provide a relative indication for cataract surgery in people with sleep impairment and depression who have prominent crystalline lens yellowing despite good high contrast visual performance. OM
References
1. Mainster MA. Violet and blue light blocking intraocular lenses: photoprotection versus photoreception. Br J Ophthalmol. 2006;90:784-92.
2. Turner PL, Mainster MA. Circadian photoreception: ageing and the eye's important role in systemic health. Br J Ophthalmol. 2008;92:1439-44.
3. Turner PL, Van Someren EJW, Mainster MA. The role of environmental light in sleep and health: Effects of ocular aging and cataract surgery. Sleep Med Rev. 2010;14:269-80.
4. Schmoll C, Lascaratos G, Dhillon B, Skene D, Riha RL. The role of retinal regulation of sleep in health and disease. Sleep Med Rev, Epub. 29 October 2010.
5. Kessel L, Lundeman JH, Herbst K, Andersen TV, Larsen M. Age-related changes in the transmission properties of the human lens and their relevance to circadian entrainment. J Cataract Refract Surg. 2010;36:308-12.
6. Hattar S, Liao HW, Takao M, Berson DM, Yau KW. Melanopsin-containing retinal ganglion cells: architecture, projections, and intrinsic photosensitivity. Science. 2002;295:1065-70.
7. Berson DM, Dunn FA, Takao M. Phototransduction by retinal ganglion cells that set the circadian clock. Science. 2002;295:1070-3.
8. Dacey DM, Liao HW, Peterson BB et al. Melanopsin-expressing ganglion cells in primate retina signal colour and irradiance and project to the LGN. Nature. 2005;433:749-54.
9. Do MT, Yau KW. Intrinsically photosensitive retinal ganglion cells. Physiol Rev. 2010;90:1547-81.
10. Mure LS, Rieux C, Hattar S, Cooper HM. Melanopsin-dependent nonvisual responses: evidence for photopigment bistability in vivo. J Biol Rhythms. 2007;22:411-24.
11. Markwell EL, Feigl B, Zele AJ. Intrinsically photosensitive melanopsin retinal ganglion cell contributions to the pupillary light reflex and circadian rhythm. Clin Exp Optom. 2010;93:137-49.
12. Brown TM, Gias C, Hatori M et al. Melanopsin contributions to irradiance coding in the thalamo-cortical visual system. PLoS Biol. 2010;8:e1000558.
13. McCarty CA, Nanjan MB, Taylor HR. Vision impairment predicts 5 year mortality. Br J Ophthalmol. 2001;85:322-6.
14. Stratmann M, Schibler U. Properties, entrainment, and physiological functions of mammalian peripheral oscillators. J Biol Rhythms. 2006;21:494-506.
15. West KE, Jablonski MR, Warfield B et al. Blue light from light-emitting diodes (LEDs) elicits a dose-dependent suppression of melatonin in humans. J Appl Physiol, Epub. 16 December 2010.
16. Thapan K, Arendt J, Skene DJ. An action spectrum for melatonin suppression: evidence for a novel non-rod, non-cone photoreceptor system in humans. J Physiol. 2001;535:261-7.
17. Brainard GC, Hanifin JP, Greeson JM et al. Action spectrum for melatonin regulation in humans: evidence for a novel circadian photoreceptor. J Neurosci. 2001;21:6405-12.
18. Mishima K, Okawa M, Shimizu T, Hishikawa Y. Diminished melatonin secretion in the elderly caused by insufficient environmental illumination. J Clin Endocrinol Metab. 2001;86:129-34.
19. Herljevic M, Middleton B, Thapan K, Skene DJ. Light-induced melatonin suppression: age-related reduction in response to short wavelength light. Exp Gerontol. 2005;40:237-42.
20. Asplund R, Lindblad BE. Sleep and sleepiness 1 and 9 months after cataract surgery. Arch Gerontol Geriatr. 2004;38:69-75.
21. Asplund R, Lindblad BE. The development of sleep in persons undergoing cataract surgery. Arch Gerontol Geriatr. 2002;35:179-87.
22. Mainster MA, Turner PL. Blue-blocking IOLs decrease photoreception without providing significant photoprotection. Surv Ophthalmol. 2010;55:272-89.
23. Mainster MA, Turner PL. Blue-blocking IOLs vs. short-wavelength visible light: hypothesis-based vs. evidence-based medical practice (in press, January). Ophthalmology. 2011.
24. Kessel L, Eskildsen L, van der Poel M, Larsen M. Non-invasive bleaching of the human lens by femtosecond laser photolysis. PLoS One. 2010;5:e9711.
25. American-Academy-of-Ophthalmology. Contrast sensitivity and glare testing in the evaluation of anterior segment disease. Ophthalmology. 1990;97:1233-7.
Patricia L. Turner, MD is a clinical associate professor and Martin A. Mainster, PhD, MD is Luther and Ardis Fry Professor Emeritus of Ophthalmology in the department of ophthalmology at the University of Kansas School of Medicine. Dr. Turner has received travel grants from Abbott Medical Optics. Professor Mainster is a consultant for Abbott Medical Optics, Iridex and Ocular Instruments. Neither has a proprietary interest in any manufacturer, patent or product. Contact them at plturnermd@att.net or mmainste@kumc.edu. |