The Expanding Role of SDOCT in Practice
Upgrades add versatility and ever-greater precision.
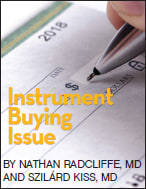
The evolution of OCT from the time-domain to spectral-domain platform was initially applauded for an increase in the resolution of acquired images. Consider that when the first commercial OCT instrument was developed in 1995, 100 A-scans per second were acquired and the axial resolution was 20 microns. The Cirrus HD-OCT instrument, released in 2007, collects 27,000 A-scans per second with an axial resolution of five microns.
However, several advantages of SDOCT beyond better axial resolution are worthy of recognition. Because the speed of acquisition has been dramatically increased by abandoning the moving reference mirror (time domain) and using Fourier domain analysis of the collected data, OCT images can now be rapidly obtained in large data cubes rather than line scans, and these cubes are now becoming the standard format for OCT image acquisition.
With significantly more high-resolution data to analyze, recent developments in OCT acquisition and analysis software are instrumental in allowing ophthalmologists to interpret the data. In most cases, these software updates allow three-dimensional data cubes to be registered, allowing for point-by-point registration and comparison over time. Finally, many SDOCT manufacturers now offer anterior segment scanning modules.
The summation of these software and hardware enhancements is that SDOCT instruments now provide the clinician with a means of performing comprehensive ophthalmic evaluation applicable to several specialties and across many regions of the eye. In this article, SDOCT applications will be reviewed for anterior segment, glaucoma and neuro-ophthalmic conditions. While this article will primarily feature OCT applications using the Cirrus HD-OCT due to the author's familiarity, similar instruments from other manufacturers either currently provide or are in development of the uses discussed below.
Retina
One recent advance in retinal OCT is the rendering of a series of conventional 2D slices of spectral domain OCT images into 3D volumetric objects. With the advent of high-speed image acquisition, 100 or more individual cross sections of the retina can be acquired in a very short amount of time. Although these slices are extremely useful individually, putting them together shows the actual structures of the retina, not just in cross-section, but also en face. This 3D reconstruction then allows for isolation, segmentation and quantification of specific retinal structures.
For example, in patients with age-related macular degeneration, drusen volume and the area of geographic atrophy can be calculated and followed in response to specific pharmacological interventions. In addition to visual acuity measurements, these measurements can serve as a surrogate endpoint both in the care of our patients and in clinical trials. Moreover, characterizing these 3D relationships may aid in how we actually treat our patients. The treatment of vitreomacular traction (with observation, surgery or pharmacological vitreolysis), for example, may be aided by calculating the area of adhesion of the vitreous to the retina.1 In patients with certain thresholds of adhesion, one treatment alternative (such as injection of microplasmin) may emerge as the best treatment recommendation. For all these relationships seen via an OCT, larger, prospective studies are obviously needed to validate the preliminary findings.
Yet another important recent advance in retinal OCT is that of enhanced depth imaging. With EDI, ocular features beyond the retinal layers (deeper than the RPE) can be clearly visualized.2 For the first time, we can obtain high-resolution images of the choroid and optic nerve using EDI OCT. Even in the two years since its first introduction by Spaide and colleagues, EDI OCT has provided some fundamental insights into such diseases as central serous chorioretinopathy, Vogt-Koyanagi-Harada disease (VKH), pathologic myopia and unexplained vision loss in elderly patients who do not have any signs of AMD. In VKH, for example, the choroidal thickness appears to be significantly thicker when compared with unaffected patients.3 Moreover, the choroidal thickness varies with VKH disease activity—becoming thicker as the inflammation returns and thinning out with treatment. EDI OCT can thus serve as a biomarker for disease activity in which potential treatment decisions are made even prior to the patient becoming symptomatic.
Glaucoma
As glaucoma is characterized as an optic neuropathy in which retinal nerve fiber layer apoptosis results in retinal nerve fiber layer atrophy, initial applications of OCT in glaucoma focused on a 360-degree circumpapillary line scan with automated segmentation of the RNFL. Because data cubes surrounding the optic nerve may now be obtained, RNFL thickness maps are now available which more intuitively demonstrate arcuate RNFL bundle defects as seen with fundus photography (Figure 1). SDOCT is probably more sensitive than time-domain OCT for the detection of glaucoma.4
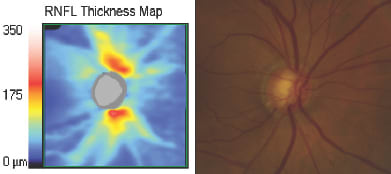
Figure 1. Characteristic superotemporal and inferotemporal RNFL defects are seen on the RNFL thickness (left) map and correspond to regions of RNFL loss on fundus photography (right).
Additionally, by using the deep retinal feature of termination of Bruch's membrane to define the optic disc rim, OCT data can be used to measure the neuroretinal rim, cup volume and other optic nerve head features.5 This approach is likely to be advantageous to confocal scanning laser ophthalmoscopy, which measures retinal surface topography only and, as such, has problems correctly identifying the optic disc rim, often requiring user modification based on stereoscopic optic nerve infection. While HRT has shown value in the determination of glaucoma progression, it may be less valuable for distinguishing glaucomatous discs from normal.6
By incorporating both neuroretinal rim and RNFL features, SD-OCT represents a platform that allows the comparison of distinct retinal and optic nerve features. For example, patients with physiological cupping who do not have glaucoma should not have RNFL abnormalities, and by assessing both structures, the specificity for glaucoma detection should be enhanced (Figure 2). Additionally, consider that when interpreting RNFL circle scans in isolation, most optic neuropathies (in addition to glaucoma) cause loss of RNFL. As discussed below, these conditions include nonarteritic ischemic optic neuropathy, compressive lesions such as tumors, and optic disc drusen (see discussion below). While a few pathologies other than glaucoma can cause cupping in addition to RNFL loss (traumatic optic neuropathy, syphilis and arteritic ischemic optic neuropathy), the coincidence of cupping and RNFL loss is a finding more specific to glaucomatous optic neuropathy than RNFL loss alone.
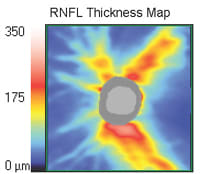
Figure 2. An RNFL thickness map with neuroretinal rim segmentation (gray) in a glaucoma suspect with a normal visual field. The image demonstrates that although the vertical CD ratio is enlarged at about 0.6, the retinal fiber thickness is normal.
Glaucoma Progression
Initial attempts to evaluate for glaucoma progression using TDOCT were somewhat limited by the lack of standard placement of the circular line scan around the optic nerve. Eccentrically placed line scans can result in misalignment of RNFL measurements and will introduce noise and registration error into the progression assessment. Volumetric OCT data cubes can be automatically aligned and compared over time.
The Cirrus HD-OCT collects a standard optic nerve cube of 200 x 200 A-scans over a 5-mm area of retina. Familiar measurements such as a circumpapillary retinal scan can then be extracted from the data cube. The resulting data cube is available for all future analyses. For example, the recently released Cirrus HD-OCT ONH module and normative database can be retrospectively applied to previously acquired optic nerve data cubes, and the newly developed ganglion cell complex analysis (not yet released) can be applied to previously acquired macular cube scans. For example, the circle scan can be repositioned at a later date and the cube of OCT data can be reanalyzed with the new circle scan placement.
Another advantage of using the data cube is the ability to ensure that circle scan and neuroretinal rim data are analyzed in a plane that is perpendicular to the peripapillary retina to control for the influence of optic nerve tilt, a feature that is not achievable using line scans. Once reliable and repeatable measurements of RNFL or neuroretinal rim parameters have been acquired, it is possible to evaluate for disease progression using event (change from baseline) or trend (linear change over time) based techniques.
Anterior Segment OCT
Another exciting development in SDOCT is the availability of non-contact, high definition quantitative anterior segment imaging. Using anterior segment OCT , the following corneal layers can be identified: tear film, epithelium, Bowman's layer and Descemet's membrane/endothelium. While the Visante OCT was the first device to image the anterior segment, recently several anterior segment SDOCT modules have become available that provide higher resolution images and are available without the purchase of an additional device.
In Figure 5, a 45-year-old man is seen to have iridolenticular synechiae and an anterior polar cataract, using ASOCT. The anterior chamber angle can be identified, and these high resolution images often allow the identification of Schlemm's canal (Figure 6).
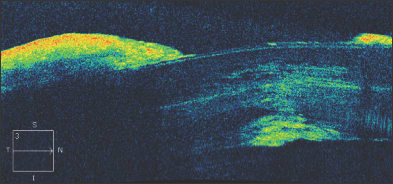
Figure 5. Anterior segment OCT demonstrating iridolenticular apposition and an anterior polar cataract.
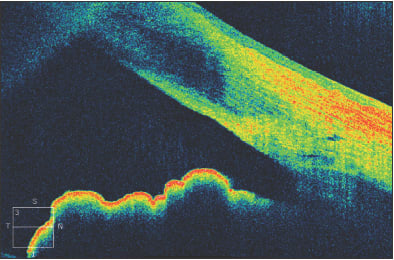
Figure 6. The anterior chamber angle in a patient with a narrow, but non-opposed, angle. Schlemm's canal can be visualized.
The results of surgical interventions can also be documented, including the position of Descemet's stripping endothelial keratoplasty buttons, glaucoma tube shunts or intraocular lenses. After penetrating keratoplasty, the graft-host interface can be evaluated as seen in this patient with a traumatic corneal laceration, resulting in an emergent penetrating keratoplasty and suboptimal graft-host alignment (Figure 7). Filtering blebs after trabeculectomy can be evaluated, and subconjunctival filtration can be demonstrated (Figure 8).
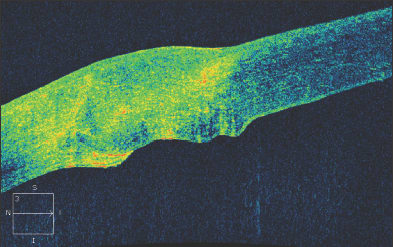
Figure 7. An irregular graft-host interface following penetrating keratoplasty.
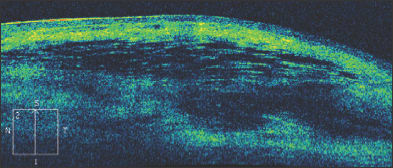
Figure 8. A filtering bleb following trabeculectomy. Fluid is present beneath the conjunctiva and Tenon's fascia.
After DSEK, the position and apposition of the donor endothelial button can be evaluated. In Figure 9a, the endothelial button can be seen to be non-adherent to the corneal stroma, with associated epithelial bullae. After several months, the endothelial button has become fully adherent, and the epithelial fluid has resolved (Figure 9b). In Figure 10, we see a patient who has undergone combined penetrating keratoplasty and tube shunt implantation, and the tube position, while somewhat anterior, is confirmed to be free of corneal touch. The graft-host junction is visualized and the corneal stroma and endothelium anterior to the tube tip appear normal.
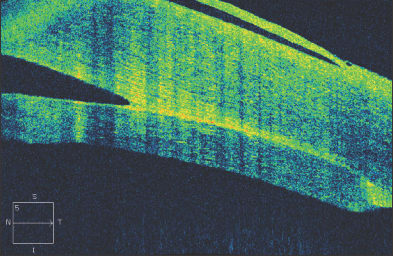
Figure 9. Shortly following Descemet's stripping endothelial keratoplasty, the endothelial button can be seen to be non-adherent to the corneal stroma, with associated epithelial bullae (Fig. 9a, above). After several months, the endothelial button has become fully adherent, and the epithelial fluid has resolved (Fig. 9b, below).
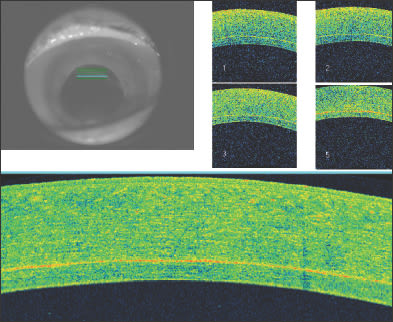
Figure 10. Anterior segment OCT of a patient who has undergone combined penetrating keratoplasty and tube shunt implantation. The tube position, while somewhat anterior, is confirmed to be free of corneal touch.
Beyond diagnosis of pathologies, anterior segment OCT can be a valuable patient educational tool, particularly in the case of anatomical narrow angle, a condition that is intrinsically difficult for patients to understand because of the complex anatomy involved. Finally, anterior segment OCT is currently being used to register corneal anatomy for the placement of surgical wounds during femtosecond cataract surgery and this application is likely to expand as femtosecond cataract surgery evolves.
Neuro-ophthalmic applications of OCT
• Neurodegenerative Diseases. Parkinson's disease, the second most common neurodegenerative disease, is a progressive disorder with selective dopaminergic neuronal loss. Unfortunately, there are currently no objective biomarkers of disease severity, progression or response to treatment. As dopaminergic neuronal cells have been identified in the inner nuclear layer and inner plexiform layers of the human retina, several groups have used spectral-domain OCT in an attempt to quantify retinal changes that may correlate with Parkinson's disease activity. Thinning of the retinal nerve fiber layer, the inner retinal layer and macular thickness have been documented in several small studies.7 Larger, prospective studies are currently underway in order to validate these preliminary findings.
Multiple sclerosis is yet another progressive neurodegenerative disease in which spectral domain OCT may find utility in quantifying disease progression and response to treatment. Histopathologic studies have reported RNFL thinning, and these changes have been correlated with disease activity and white matter lesion volume on magnetic resonance imaging. Several smaller studies have shown that OCT may be surrogate for multiple sclerosis disease activity, specifically changes in the retinal nerve fiber layer and macular thickness measurements.8 Interestingly, these changes appear to be independent of any documented history of optic neuritis. Large prospective studies are currently under way in which OCT imaging is being used as a secondary endpoint to measure response in specific treatment protocols.
• Structure-to-structure comparisons. The use of combined RNFL and optic disc parameters using SDOCT can provide further insights by allowing evaluation of structure-to-structure comparisons of the neuroretinal rim and RNFL.9 Consider that in glaucoma, regions of optic nerve atrophy correspond to specific regions of neuroretinal rim deterioration. However, in other optic neuropathies, different relationships may exist between the RNFL and the neuroretinal rim. In optic disc drusen (Figure 3), the RNFL is atrophic and the neuroretinal rim is typically thicker than average, while in optic disc edema (Figure 4), both the RNFL and neuroretinal rim are thicker than average. Finally, conditions such as nonarteritic ischemic optic neuropathy may demonstrate RNFL loss in the presence of a normal neuroretinal rim. Finally, physiologic cupping (Figure 2) may be defined as the presence of a narrow neuroretinal rim with normal RNFL.
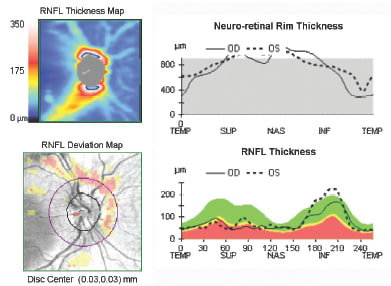
Figure 3. HD-OCT of the RNFL and neuroretinal rim in a patient with optic disc drusen and an inferior visual field defect. Note the superior RNFL loss seen on the RNFL thickness and deviation maps in the presence of a greater than average neuroretinal rim thickness.
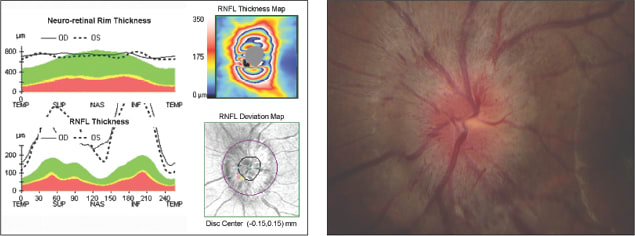
Figure 4. Optic disc edema as seen by HD-OCT (left). The RNFL and neuroretinal rim are both thicker than average. A clinical photo (right) is consistent with optic nerve edema.
Conclusion
In summary, SDOCT applications are rapidly expanding. Once a tool used mainly to enhance retinal diagnosis, SDOCT is now vital to the diagnosis and management of retinal, glaucomatous, anterior segment and neuro-ophthalmic pathologies. Knowledgeable clinicians will familiarize themselves with the available software enhancements to analyze cubes of OCT data and to evaluate for disease progression. With time, it is likely that the applications for SDOCT technologies will expand and that SDOCT will become an instrumental part of the evaluation of many diverse ophthalmic pathologies. OM
References
1. Aaker G, Gracia L, Myung J, Borcherding V, Banfelder J, D'Amico DJ, Kiss S. Three-Dimensional Reconstruction and Analysis of Vitreomacular Traction: Quantification of Cyst Volume and Vitreoretinal Interface Area. In Press, Archives of Ophthalmology.
2. Margolis R, Spaide RF. A pilot study of enhanced depth imaging optical coherence tomography of the choroid in normal eyes. Am J Ophthalmol. 2009 May;147(5):811-5.
3. Fong AH, Li KK, Wong D. Choroidal evaluation using enhanced depth imaging spectral-domain optical coherence tomography in Vogt-Koyanagi-Harada disease. Retina. 2011 Mar;31(3):502-9.
4. Cho JW, Sung KR, Hong JT, Um TW, Kang SY, Kook MS. Detection of glaucoma by spectral domain-scanning laser ophthalmoscopy/optical coherence tomography (SD-SLO/OCT) and time domain optical coherence tomography. J Glaucoma. 2011;20:15-20.
5. Mwanza JC, Oakley JD, Budenz DL. Ability of Cirrus HD-OCT Optic Nerve Head Parameters to Discriminate Normal from Glaucomatous Eyes Ophthalmology 2011;118:241–248.
6. Leung CK, Ye C, Weinreb RN, Cheung CY, Qiu Q, Liu S, Xu G, Lam DS. Retinal nerve fiber layer imaging with spectral-domain optical coherence tomography a study on diagnostic agreement with Heidelberg Retinal Tomograph. Ophthalmology. 2010;117:267-74.
7. Aaker GD, Myung JS, Ehrlich JR, Mohammed M, Henchcliffe C, Kiss S. Detection of retinal changes in Parkinson's disease with spectral-domain optical coherence tomography. Clin Ophthalmol. 2010 Dec 6;4:1427-32.
8. Khanifar AA, Parlitsis GJ, Gauthier SA, Kiss S. Evaluating retinal abnormalities in patients with multiple sclerosis. Arch Neurol. 2010 Aug;67(8):1035.
9. Suh MH, Kim SH, Park KH, Kim SJ, Kim TW, Hwang SS, Kim DM. Comparison of the correlations between optic disc rim area and retinal nerve fiber layer thickness in glaucoma and nonarteritic anterior ischemic optic neuropathy. Am J Ophthalmol. 2011;151:277-86.
![]() | Nathan Radcliffe, MD (pictured), a glaucoma specialist, is an assistant professor of ophthalmology and director of the Glaucoma Service at Weill Cornell Medical College and New York-Presbyterian Hospital in New York. Retina specialist Szilárd Kiss, MD, is director of clinical research at Weill Cornell Medical College. |